In 1878, Eadweard Muybridge used his state-of-the-art cameras to capture photographic images of a galloping horse. The celebrated pictures unambiguously revealed that there are moments during a gallop when all four hooves are off the ground. Muybridge's iconic photographic series has come to symbolize the ability of optical technological improvements to uncover aspects of nature that have remained hidden from view. In this case, the cameras, equipped with fast shutters, were able to bring into focus information that would otherwise be invisible to the human eye. A new area of exploration had begun, spurred by the newly acquired speed of photography, into nature's dynamics. From unveiling the movement of humans to intercepting the flapping wings of a flying bird, as long as the image-capturing speed exceeds the motion of the subjects the dynamic targets could be frozen in time as photographic stills. The criteria for creating snapshots of moving humans, horses, and birds in our macroscopic world also apply to visualizing the microscopic world of cells and biological tissues. Cells, like humans, are in constant motion, displaying dynamics on a wide variety of spatial and temporal scales. The submicrometer resolution provided by the optical microscope has cleared the way for zooming into the spatial scales relevant to cell and tissue biology. Using the fast cameras of the day, many cellular processes and dynamic changes in live tissues can be “caught in the act.” Nonetheless, imaging speed alone is not enough. Simple photography does not suffice to selectively visualize the molecular components that govern biological processes. Specific contrast is needed that selectively highlights the molecules. Among the molecular specific light-matter interactions, the Raman process has gained a lot of prominence because of its ability to probe endogenous molecules without the use of labels. The Raman interaction seems to be a perfect match for optical microscopy of biological samples. The vibrational contrast enables the selective probing of a wide variety of molecules that are essential to cellular life, ranging from lipids, water and proteins, to carbohydrates and nucleic acids. The vibrational method is thus able to investigate the signatures of healthy and diseased tissue by directly visualizing the tissue's most essential ingredients. In addition, external agents such as topically applied drugs can be seen through select molecular vibrations, which is directly relevant to pharmaceutical research. This label-free contrast is accompanied by another unique aspect of the Raman interaction: the high spatial resolution provided by the visible and near-infrared excitation light, which is many times higher than in competing vibrational imaging techniques based on infrared excitation. The combination of high resolution and molecular contrast has moved Raman techniques into the biomedical spotlight. Despite these virtues, the Raman technique suffers from an important caveat that limits its impact on biomedical imaging. The spontaneous Raman interaction is weak, yielding insufficient photons for fast imaging applications. Although superb for chemical mapping of motionless samples, the spontaneous Raman microscope is unable to muster up the speed for capturing stills of live tissues and cells. To move the Raman technique into the realm of imaging of live biology, a faster ‘camera’ is needed that is able to take vibrational snapshots at a much higher rate. From this perspective, it is not surprising that the much higher image acquisition times offered through nonlinear Raman techniques have made a big splash in the field of biomedical imaging, as it has firmly established Raman vibrational imaging as a noninvasive and label-free tool for live tissues and cells. The field of nonlinear Raman microscopy is a field in motion. From the early days of the proof-of-principle coherent anti-Stokes Raman scattering (CARS) microscope in the early 1980s,1 to the 3-D CARS microscope with simplified collinear excitation geometry in 1999,2 the subsequent improvements in speed and contrast in the early years of the twenty-first century,3, 4 and the development of the background-free stimulated Raman scattering (SRS) imaging microscope,5 the field has always been driven by technological innovation. The push for better biomedical microscopes has brought together optical theorists, laser physicists, electrical engineers, microscope builders, fiber crafters, lens designers, and software programmers, who have jointly raised the performance of coherent Raman scattering (CRS) microscopes. Similar to Muybridge's advanced cameras, these technological improvements have continuously expanded the horizon of imaging applications. CRS microscopy enables the study of important aspects of tissue biology that have previously remained out of reach. The impact of CRS microscopy can already be heard loud and clear in the area of lipid biology and lipid-related diseases. The fast imaging capabilities of the CRS microscope have enabled researchers to look at, for instance, intestinal lipid absorption and myelin-related neurodegenerate diseases in live animal models, in addition to lipid metabolism studies in live microorganisms.6 Such studies were unthinkable with conventional Raman techniques. But the ramifications of CRS microscopy are by no means limited to the field of lipid biology. For example, real-time CRS imaging has also opened the door toward the direct visualization of topically applied agents to human skin, a capability new to the field of biomedical imaging and with potentially large implications for drug development. In addition, CRS microscopy has shown promise as a fast histopathological tool. By providing contrast similar to what can be obtained with conventional histochemical staining protocols, CRS methods are offering an in vivo alternative to standard invasive histological methods. Broadband CRS methods add further colors to this palette, and show promise as rapid tools for chemical mapping of cells and tissues with an expanded sensitivity to a larger set of biochemical compounds. With important biomedical applications coming to fruition, the mutual interaction between CRS technique development and applications has become even stronger. The prospect of using CRS imaging as a diagnostic tool in a clinical setting has spurred new advances in compact ultrafast lasers and miniaturization of nonlinear Raman probes. Important applications, such as intravital imaging of lipid-rich plaques in arterial disease, form a constant driving source for further technological improvement and are a constant reminder of the vast implications of the coherent Raman interaction. In this special section, we have tried to capture some of the diversity and dynamism of the field of coherent Raman imaging. The papers in this section cover a wide variety of topics. Several papers illuminate exciting developments in light-source technologies, microscope design, multimodality integration, and real-time scanning techniques. This issue also contains papers that discuss new multivariate analysis approaches for CARS imaging applications. The technical issues concerning imaging in turbid media are found herein as well, along with detailed CARS imaging studies of bioengineered tissues. Finally, it includes several exciting CRS biological imaging studies, ranging from lipid interactions in monocytes to imaging applications in neurodegenerate disease, arterial disease, and brain tumor analysis. Together, these papers sketch a timely portrait of the state-of-the-art of the CRS technology and illustrate its role as a young, yet vital player in the field of biomedical imaging. ReferencesM. Duncan, J. Reintjes, and
T. J. Manuccia,
“Scanning coherent anti-Stokes Raman microscope,”
Opt. Lett., 7
(8), 350
–352
(1982). https://doi.org/10.1364/OL.7.000350 Google Scholar
A. Zumbusch, G. Holtom, and
X. S. Xie,
“Vibrational microscopy using coherent anti-Stokes Raman scattering,”
Phys. Rev. Lett., 82 4142
–4145
(1999). https://doi.org/10.1103/PhysRevLett.82.4142 Google Scholar
J. X. Cheng,
“Coherent anti-Stokes Raman scattering microscopy,”
Appl. Spectros., 61
(2), 197
–208
(2007). https://doi.org/10.1366/000370207781746044 Google Scholar
C. L. Evans and
X. S. Xie,
“Coherent anti-Stokes Raman scattering microscopy: chemical imaging for biology and medicine,”
Annu. Rev. Anal. Chem., 1 883
–909
(2008). https://doi.org/10.1146/annurev.anchem.1.031207.112754 Google Scholar
C. W. Freudiger,
“Label-free biomedical imaging with high sensitivity by stimulated Raman scattering microscopy,”
Science, 322 1857
–1861
(2008). https://doi.org/10.1126/science.1165758 Google Scholar
T. T. Le, S. Yue, and
J. X. Cheng,
“Shedding new light on lipid biology with coherent anti-Stokes Raman scattering microscopy,”
J. Lipid Res., 51 3091
–3102
(2010). https://doi.org/10.1194/jlr.R008730 Google Scholar
|
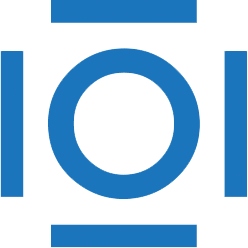
CITATIONS
Cited by 6 scholarly publications.
Raman spectroscopy
Biomedical optics
Chromium
Coherence imaging
Tissues
Microscopes
Biology